▶️ Watch Now: What Is Electromagnetic Induction? How Faraday’s Law Works
What Is Electromagnetic Induction?
Definition and Basic Concept
Electromagnetic induction refers to the phenomenon where a changing magnetic field generates voltage and current in a conductor. This principle was first discovered in 1831 by British scientist Michael Faraday, who demonstrated that a moving or varying magnetic field can “induce” an electric current in a wire. It is the foundational concept behind modern generators and transformers.
In simple terms, when a magnet moves toward or away from a coil, or when a coil passes through a magnetic field, a voltage is created in the coil. If the circuit is closed, current flows—this is electromagnetic induction.
How Magnetism Creates Electricity
The key to electromagnetic induction lies in the change in magnetic flux—the total number of magnetic field lines passing through the conductor’s area. When this magnetic flux changes, an induced voltage is generated in the conductor.
For example, if a magnet moves quickly through a coil, the rapid change in magnetic field results in a larger induced voltage. But if the magnet is still, even if it’s inside the coil, no voltage is produced.
Real-Life Examples of Electromagnetic Induction
- Generators: Convert motion into electricity by rotating magnets or coils.
- Induction cooktops: Use induced currents (eddy currents) to heat cookware directly.
- Wireless charging: Transfers energy to devices like smartphones via inductive coils.
- Card readers and access systems: Read information from cards using electromagnetic induction.
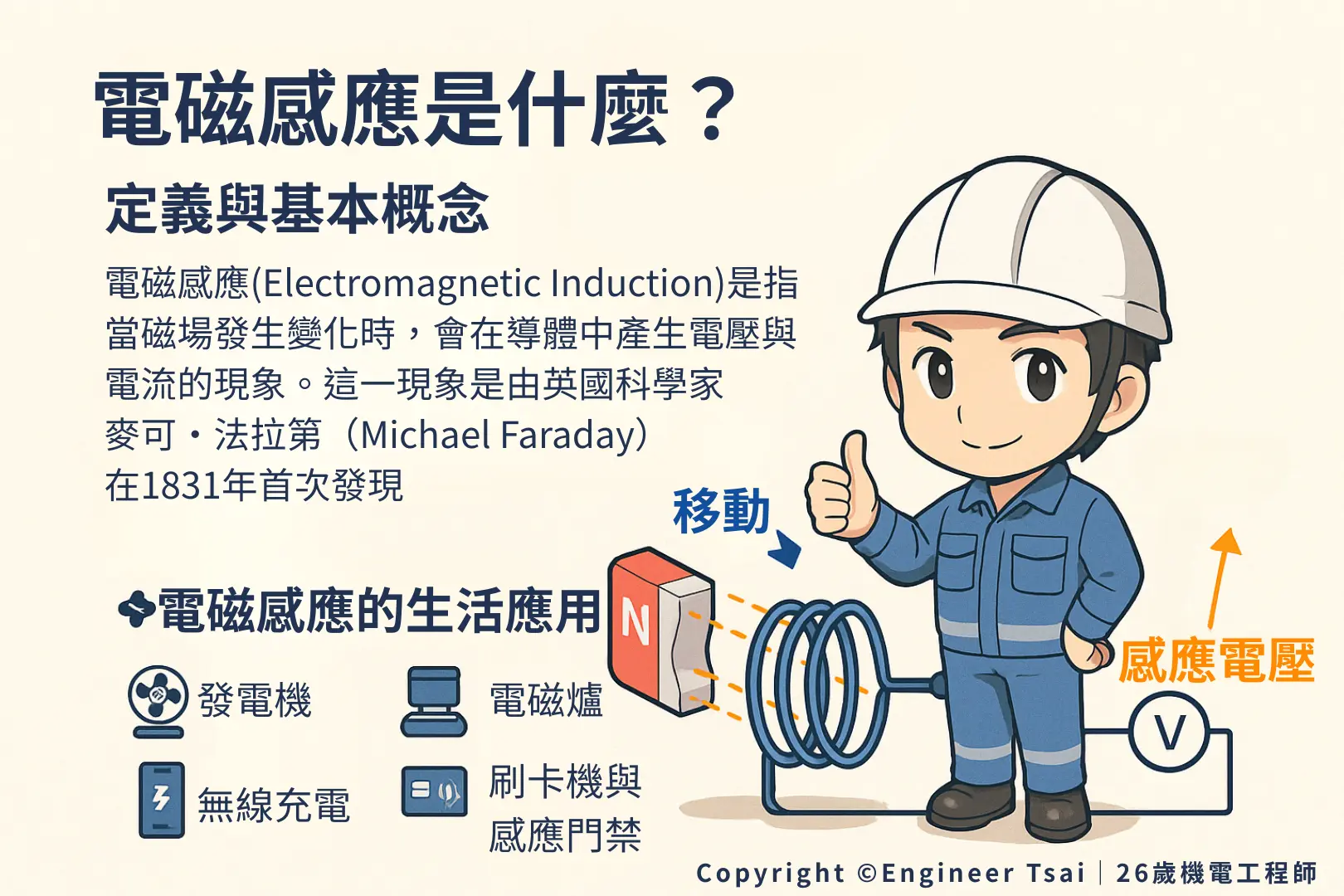
Faraday’s Law of Electromagnetic Induction
Faraday’s Law: Equation and Explanation
Faraday’s Law of Electromagnetic Induction states that:
The magnitude of the induced voltage is proportional to the rate of change of magnetic flux.
The mathematical expression is:
E = -dΦ/dt
Where:
- E = Induced voltage (Volts)
- Φ (Phi) = Magnetic flux (Webers)
- dΦ/dt = Rate of change of magnetic flux
- The negative sign reflects Lenz’s Law, indicating that the induced voltage opposes the change in flux that caused it.
Understanding Magnetic Flux
Magnetic flux is the total magnetic field passing through a given area. The formula is:
Φ = B × A × cos(θ)
Where:
- B = Magnetic field strength (Teslas)
- A = Area of the conductor (square meters)
- θ = Angle between the magnetic field and the normal (perpendicular) to the surface
Changes in magnetic field strength, conductor area, or the angle between them will alter the magnetic flux—and thus induce a voltage.
Conditions for Induction & Physical Meaning
To induce voltage, at least one of the following conditions must be met:
- Change in magnetic field strength (e.g., moving a magnet)
- Change in area (e.g., stretching or expanding a loop)
- Change in angle (e.g., rotating the coil)
Each of these conditions represents a different way to vary magnetic flux. By manipulating these variables, we can generate or predict induced voltage.
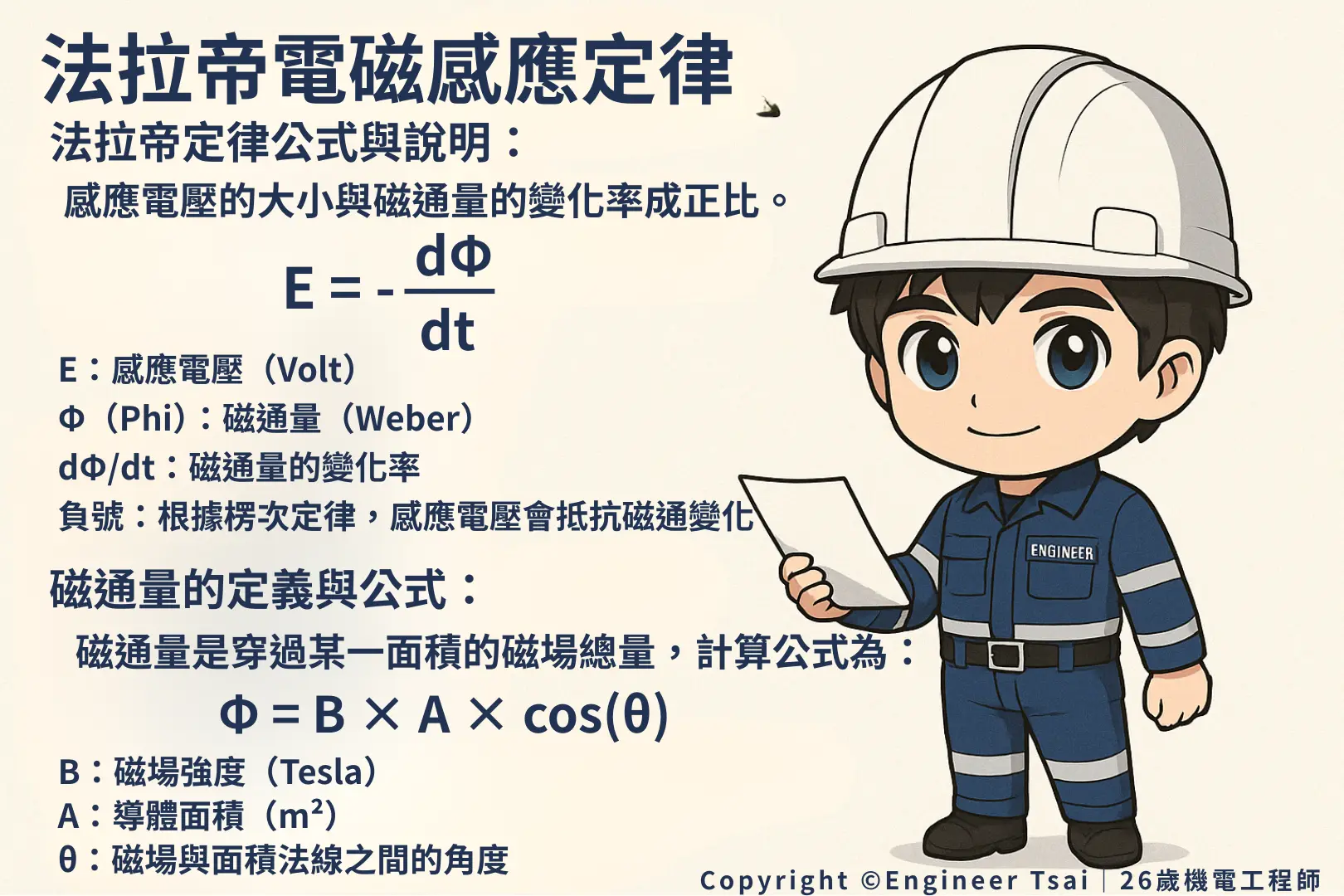
Lenz’s Law: The Direction of Induced Current
Origin and Explanation of Lenz’s Law
Lenz’s Law complements Faraday’s Law by describing the direction of the induced current. It states:
The induced current will create a magnetic field that opposes the change in magnetic flux that caused it.
In simple terms, the circuit doesn’t passively accept the change in magnetic flux—it resists it. This resistance reflects the law of conservation of energy: energy cannot be created from nothing.
How Induced Current Resists Change
Here are two common examples:
- If a magnet approaches a coil, increasing magnetic flux, the induced current generates a magnetic field opposite to the magnet’s—trying to slow the change.
- If a magnet moves away, decreasing flux, the coil produces a magnetic field in the same direction—trying to “keep” the flux.
This behavior ensures that the system doesn’t violate physical laws, such as producing infinite energy.
The Connection to Energy Conservation
At its core, Lenz’s Law preserves energy balance. If the induced current didn’t oppose the change in flux, we could create perpetual motion machines—which are physically impossible. Thus, the direction of induced current must always act to oppose the source of change, ensuring that the system remains stable and controllable.
Real-World Applications of Electromagnetic Induction
🔹 Principle Behind Generators
Whether powered by fossil fuels, water, or wind, modern generators all rely on electromagnetic induction. When a coil rotates within a magnetic field—or when a magnetic field moves through a stationary coil—changing magnetic flux is produced, which induces an alternating voltage. This voltage drives current, delivering electricity to homes and cities.
In short: Moving magnets = making electricity!
🔹 Transformer Coupling
Transformers don’t generate power—they transfer it through electromagnetic induction. An AC current in the primary coil produces a changing magnetic field. This changing field is picked up by the secondary coil, inducing a new voltage. By adjusting the coil turn ratio, we can step voltage up or down—crucial for power distribution and electronics.
🔹 Wireless Charging & Inductive Power Transfer (IPT)
Devices like wireless charging pads for phones or toothbrush charging bases use coils to create a magnetic coupling between two sides, transferring energy without physical contact. This principle is now used in EV charging stations and implantable medical devices as well.
🔹 Inductive Braking & Heating
- Inductive braking: Used in maglev trains or exercise bikes, where electromagnetic forces create drag without physical contact.
- Induction heating: Magnetic fields generate eddy currents inside metal, heating it efficiently. This is common in induction cooktops and industrial heat treatment.
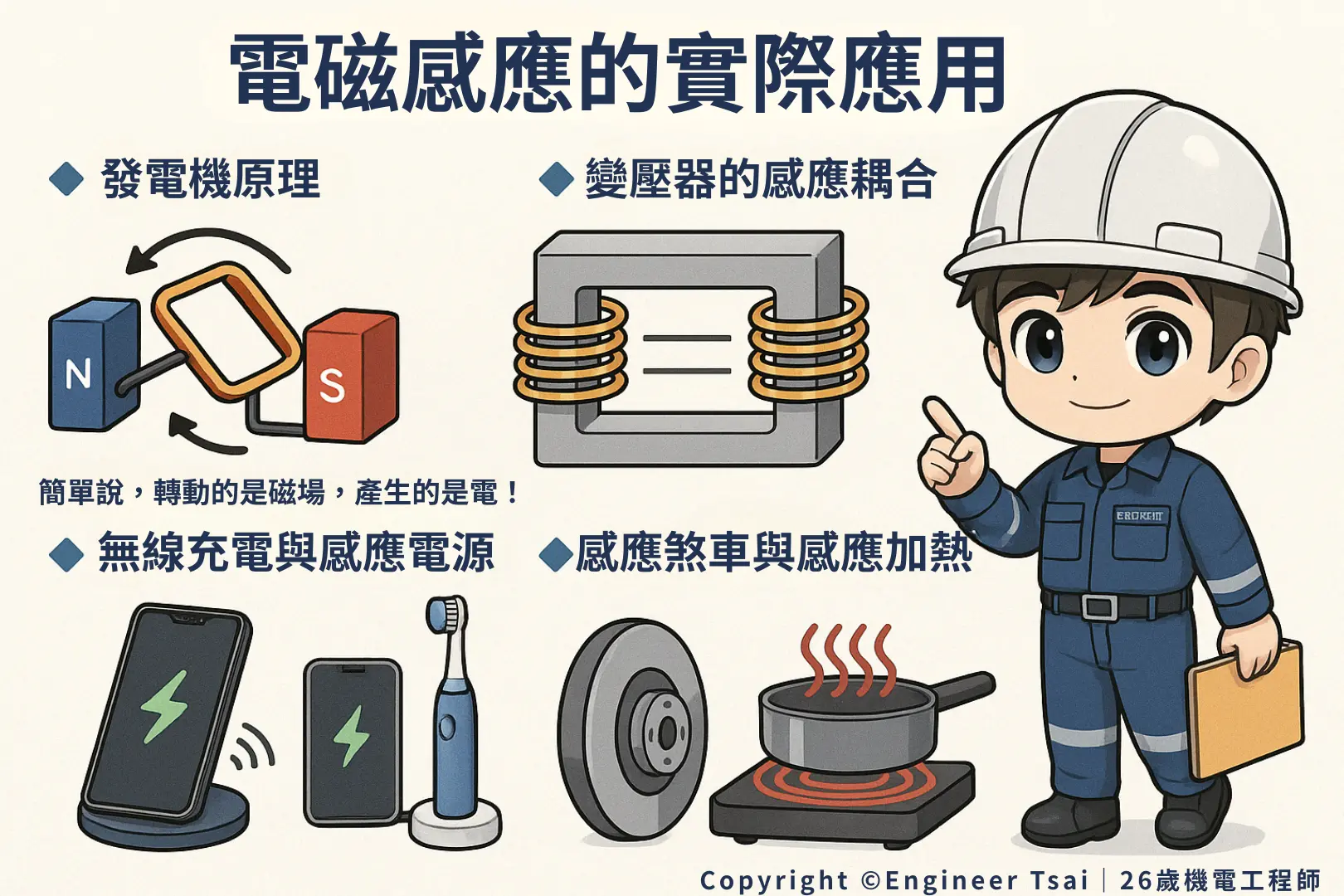
Experimental Observation: Simple Electromagnetic Induction
📌 Materials and Steps
What you need:
- A coil (several turns of insulated copper wire)
- A small magnet
- A voltmeter (or a multimeter set to measure voltage)
Steps:
- Connect the voltmeter to both ends of the coil.
- Quickly insert or remove the magnet through the coil.
- Observe the changes in the voltmeter reading.
🔎 How Induced Voltage Is Generated
Here’s what you’ll notice:
- When the magnet moves, the voltmeter needle jumps—indicating that a voltage has been induced.
- The faster the movement or the more turns in the coil, the greater the induced voltage.
- If the magnet is stationary, no voltage is produced.
This simple experiment clearly demonstrates the core idea behind Faraday’s Law: changing magnetic fields induce voltage.
✅ Key Results and Takeaways
- It’s not the presence of the magnet that matters—it’s the change in the magnetic field.
- The direction of the induced voltage changes depending on whether the magnet is moving in or out. This is a direct reflection of Lenz’s Law.
Why Electromagnetic Induction Prefers AC
Why Alternating Current (AC) Induces Voltage, But DC Doesn’t
Direct current (DC) produces a steady magnetic field that doesn’t change over time—so it can’t continuously induce voltage.
On the other hand, alternating current (AC) constantly changes direction and magnitude, creating an oscillating magnetic field. This constant change is exactly what electromagnetic induction needs to work.
Alternating Magnetic Fields and Sine Waves
When a coil rotates at a constant speed within a magnetic field, the magnetic flux changes periodically, producing a sine wave–shaped voltage. This is how AC is generated in power plants—and one of the key reasons why most power systems use alternating current.
Generator vs. Motor: Two Sides of the Same Coin
- Generator: Converts mechanical energy into electrical energy. Motion in a magnetic field induces voltage.
- Motor: Converts electrical energy into mechanical energy. Current in a coil produces magnetic force, causing rotation.
They use similar structures but operate in opposite directions—one creates electricity, the other creates motion!
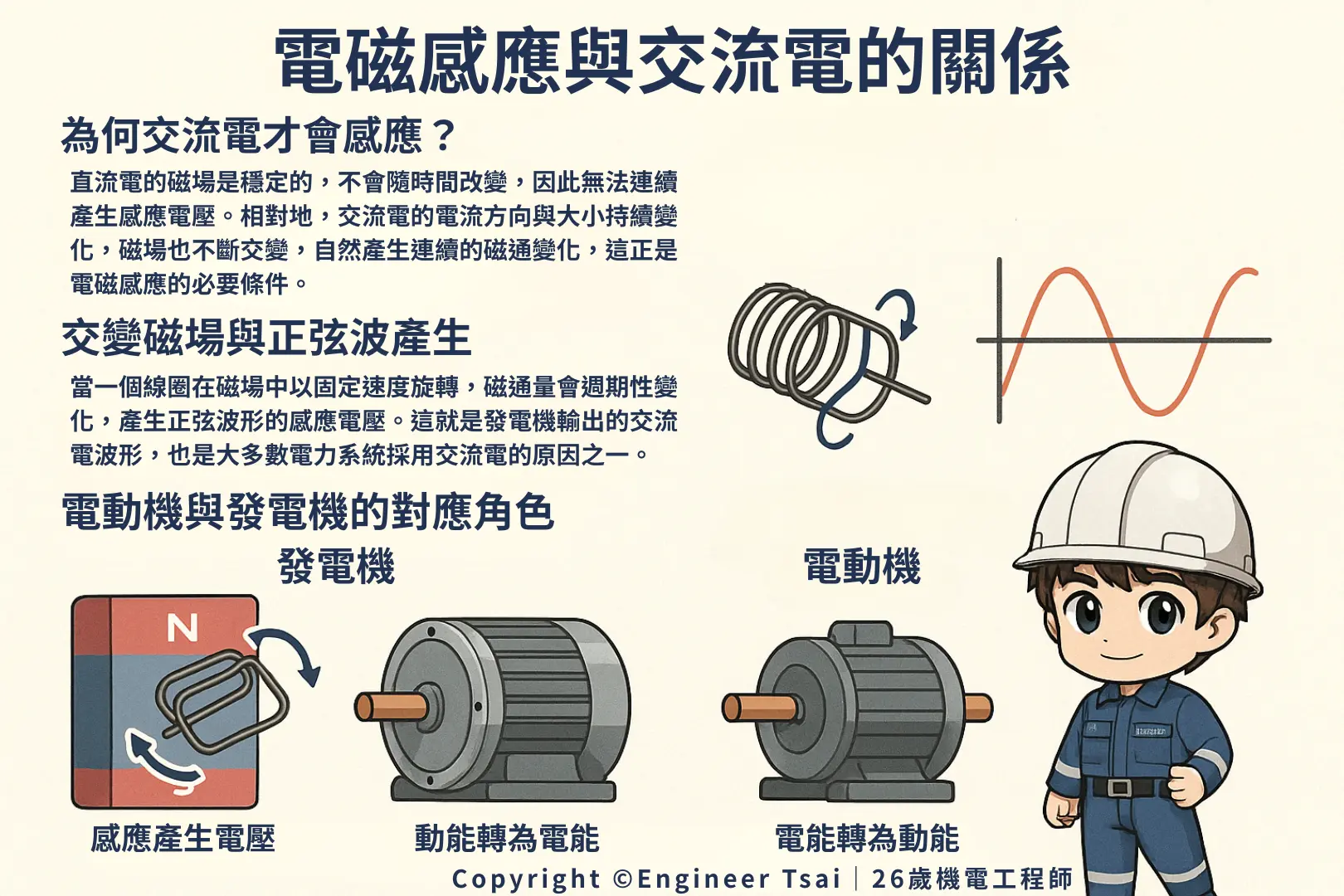
Eddy Currents and Induction Heating Technology
The Formation and Function of Eddy Currents
When a conductor is exposed to a changing magnetic field, circular loops of electric current are induced within it. These loops are known as eddy currents. As these currents flow inside the conductor, they generate heat due to electrical resistance.
While eddy currents can cause energy losses in some situations (e.g., in transformers), they are harnessed effectively in induction heating systems as a clean and efficient way to generate heat—especially for metals.
Applications of Induction Heating
Induction heating makes use of eddy currents to heat metal objects without direct contact. Common applications include:
- Induction cooktops: Heating metal pots and pans using magnetic fields.
- Industrial heat treatment: Melting, hardening, or annealing metal parts.
- Medical equipment: Flame-free sterilization tools in dentistry.
- Induction welding and cutting: Non-contact processes for metal joining and shaping.
Advantages, Drawbacks, and Efficiency
Advantages:
- Contactless and flameless, making it safe and clean.
- Fast heating and high energy efficiency.
- Precise control over heating zones and temperatures.
Drawbacks:
- Works only with materials that are electrically conductive and/or magnetic.
- Higher system costs due to specialized components.
- Potential for electromagnetic interference (EMI) from high-frequency magnetic fields.
Design Considerations and Losses in Inductive Systems
When designing induction-based devices, it’s crucial to minimize energy losses, which mainly come from:
- Coil resistance (copper loss): Heat loss due to current passing through the wire.
- Magnetic flux leakage: Some magnetic field lines may escape and not contribute to induction, lowering overall efficiency.
Key design strategies include:
- Using high-conductivity materials like oxygen-free copper to reduce resistance.
- Designing multi-layer or toroidal coils to better focus the magnetic field.
- Applying laminated iron cores or magnetic materials to limit flux leakage.
The Role of Frequency and Magnetic Materials
- Higher frequencies improve induction efficiency but also increase eddy current loss and potential EMI.
- Choosing the right magnetic materials (e.g., ferrites, silicon steel) affects how well magnetic fields are concentrated and eddy currents are controlled—both of which impact performance.
How to Improve Induction Efficiency
- Use conductors with low resistance to minimize internal heat loss.
- Optimize coil geometry for better magnetic field focus.
- Choose materials that guide and contain magnetic fields effectively.
- Match frequency and field variation rate to the specific application.
Future Trends in Electromagnetic Induction
🔋 Wireless Charging for Electric Vehicles
As EV adoption grows, wireless charging is becoming a key innovation. Simply parking over a charging pad enables high-power energy transfer through inductive coupling—no cables needed. This boosts convenience and opens the door to automated charging systems.
🧲 Superconducting Sensors and Medical Applications
Combining electromagnetic induction with superconductors allows for highly sensitive magnetic field detection, enabling:
- MRI (Magnetic Resonance Imaging)
- Brainwave sensing and neural monitoring
- Non-contact vital sign measurement systems
⚡ Energy Harvesting for Wearables and IoT
Small electromagnetic systems can harvest energy from body motion or ambient magnetic fields. Applications include:
- Smart textiles and self-powered IoT devices
- Wearable health monitors
- Battery-free sensors
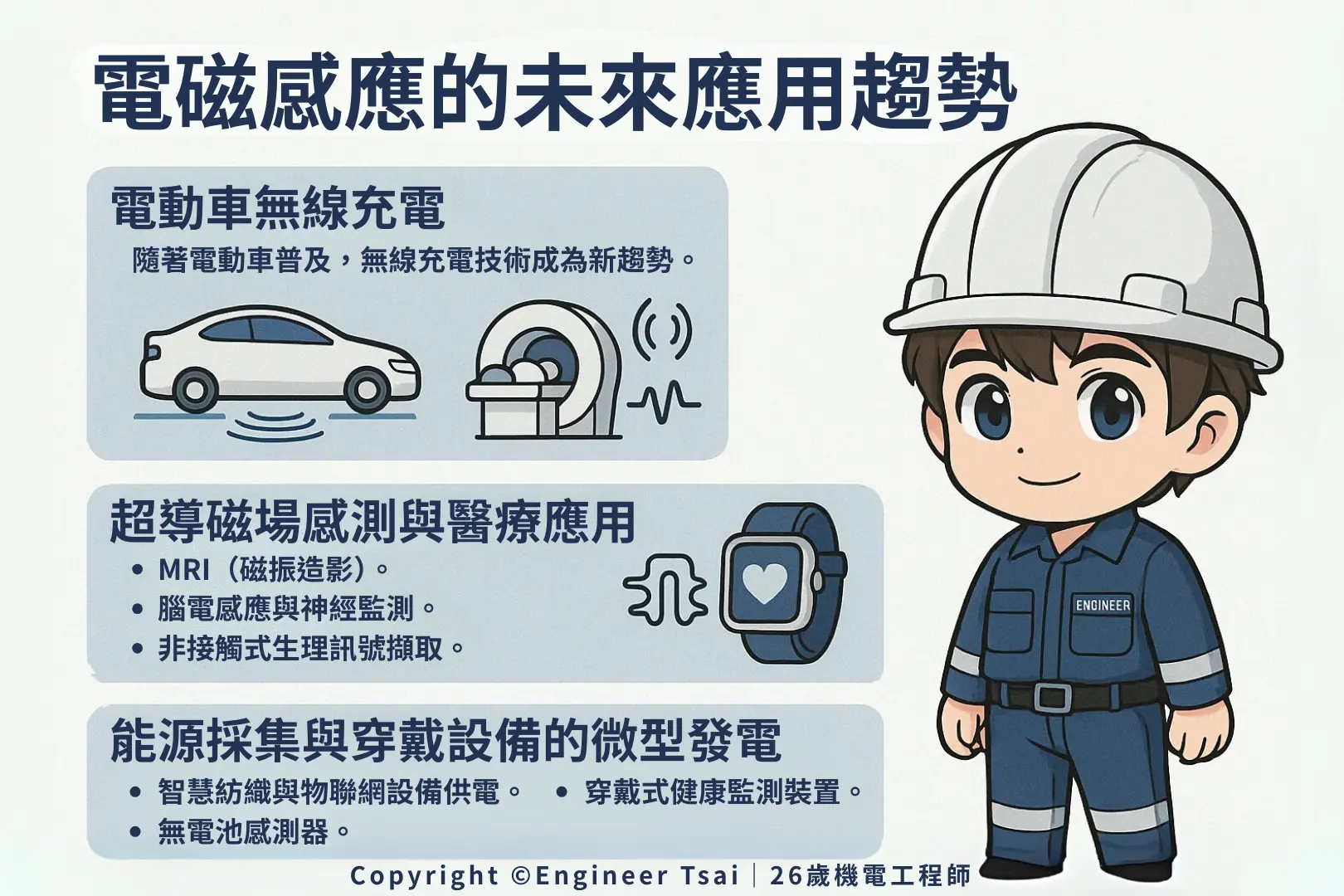
Conclusion and Further Reading
Electromagnetic induction isn’t just a law in a physics textbook—it’s a foundational principle behind modern electricity and communication. From generators and transformers to wireless charging, it powers much of our daily life. Understanding how electromagnetic induction works helps us design safer, more efficient electrical systems for the future.
📌 Further Reading
🔹 “Magnetic Fields and Electricity: The Hidden Force Powering Modern Technology”
Explore how magnetic fields and electric currents influence one another—laying the groundwork for understanding induction.
🔹 “Transformer Voltage Step-Up & Step-Down: What You Need To Know”
Learn how transformers use electromagnetic induction to step voltage up or down for transmission and usage.
🔹 “How Does Wireless Charging Work? The Electromagnetic Induction Behind It” (Coming soon)
A deep dive into the technology behind wireless charging for phones and EVs, including coil design and energy transfer.
🔹 “10 Everyday Applications of Electromagnetic Induction” (Coming soon)
From credit card readers to induction cooktops—discover the surprising ways induction shapes your daily life.